|
 |
AUTONOMIC NERVOUS SYSTEM
Fortunately
the body's vital functions are regulated automatically and
require no conscious effort on our part. The autonomic nervous
system (ANS) is to a large extent responsible for automatically
and subconsciously regulating the cardiovascular, renal,
gastrointestinal, thermoregulatory, and other systems, in order
to enable the body to meet the continual and ever-changing
stresses to which it is exposed.
Autonomic
nerve fibers innervate cardiac muscle, smooth muscle, and
glands. Through these fibers the ANS plays a role in regulating
(1) blood pressure and flow, (2) gastrointestinal movements and
secretions, (3) body temperature, (4) bronchial dilation, (5)
blood glucose levels, (6) metabolism, (7) micturition and
defecation, (8) pupillary light and accommodation reflexes, and
(9) glandular secretions, just to name a few.
A muscle or
gland innervated by autonomic fibers is called an effector
organ. If the autonomic nerve fibers to an effector organ are
cut, the organ may continue to function, but will lack the
capability of adjusting to changing conditions. If the autonomic
nerve fibers to the heart are cut, the heart will continue to
beat and pump blood normally, but its ability to increase
cardiac output under stress will be seriously limited. In a very
real sense, the ANS bestows on the vital functions of the body
the capability of adjusting activity levels to meet
ever-changing needs.
Anatomically and functionally, the autonomic nervous system is
made up of two subdivisions: the sympathetic system with
long-lasting and diffuse effects, and the parasympathetic system
with more transient and specific effects. In either case the
nerve fibers of the ANS are motor only, and represent the
general visceral efferent (GVE) fibers of the cranial and spinal
nerves.
THE
SYMPATHETIC OUTFLOW
The nerve
fibers which comprise the sympathetic system originate in the
intermediolateral horn (lamina VII) of the gray matter in all
twelve thoracic and the first two lumbar segments of the spinal
cord. The axons of these GVE fibers travel through the anterior
horn and exit the cord in the anterior root before entering the
spinal nerve. While the general somatic efferent (GSE) fibers
(alpha and gamma motor neurons of the anterior horn) continue in
the spinal nerve trunks to innervate skeletal muscle fibers and
muscle spindles, almost all of the GVE fibers leave the spinal
nerve trunks to enter sympathetic ganglia via a thin arm, the
white ramus (Figs-1, 2, and 3).
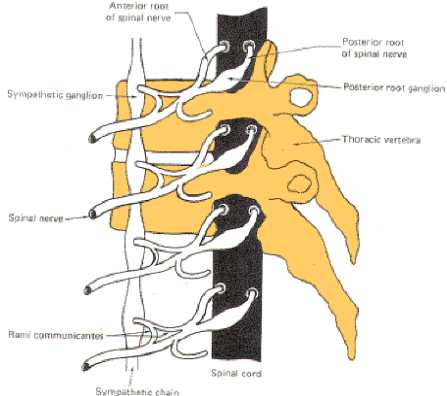 |
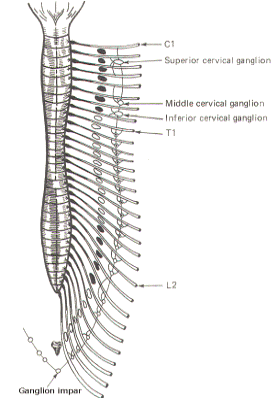 |
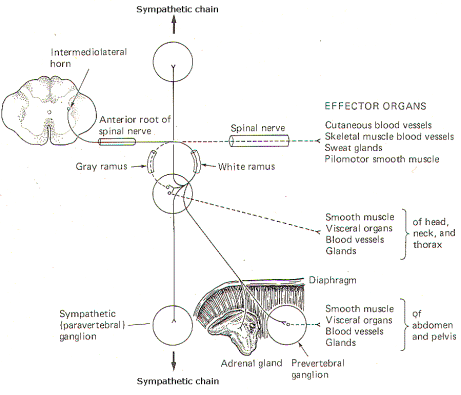 |
Fig-1: The sympathetic ganglia
associated with spinal nerves T1 through L2 are
connected to the nerve by two arms, the rami
communicantes albicans and gresium |
Fig-2: There are no rami albicans
above T1 and below L2. The gray rami send connections to
all 31 nerves. |
Fig-3: The sympathetic outflow. The
preganglionic neurons originate in the
intermediolateral horn of the spinal cord, between T1
and L2 |
The
sympathetic ganglia lie close to the vertebral bodies and are
also known as paravertebral ganglia. They are strung together to
form a sympathetic or paravertebral chain. There are two of
these chains, one on either side of the vertebral column
connected in front of the coccyx by the single ganglion impar
(Fig-2).
Some of the
fibers from nerve cells within the ganglia return to the spinal
nerve trunk via a gray ramus. The fibers traveling through the
white rami are myelinated while those in the gray rami are not,
and this fact is responsible for their respective names. Each of
the twelve thoracic and first two lumbar nerves is in contact
with a paravertebral ganglion via a white and gray ramus.
However, there are three ganglia in the chain above the thoracic
region as well as several below L2 (Fig. 14-2). Each of these
additional ganglia is connected to a spinal nerve by a single
gray ramus.
The
superior, middle, and inferior cervical ganglia probably
represent the fusion of smaller individual cervical ganglia.
These three send gray rami to all eight cervical spinal nerves.
The superior cervical ganglion sends to the first four cervical
nerves, the smaller middle cervical ganglion supplies the next
two, and the large inferior cervical ganglion projects a gray
ramus to the seventh and eighth cervical nerves. Similarly, a
variable number of ganglia (four to eight) below L2 send gray
rami to all of the spinal nerves below this level. Consequently,
all 31 pairs of spinal nerves are in contact with the
sympathetic chain and carry fibers of the sympathetic system.
This is an important feature, enabling those effector organs
which are innervated only by spinal nerves (cutaneous and
skeletal muscle blood vessels, sweat glands, and pilomotor
smooth muscle) to receive sympathetic input.
In addition
to the paired paravertebral ganglia, there are several unpaired
prevertebral ganglia in the abdomen and pelvis. They also playa
role in the sympathetic outflow. Figure 14-3 illustrates the
many possible ways by which the sympathetic system innervates
its effector organs.
There is
always a two-neuron link to each effector organ innervated. with
the single exception of the adrenal medulla. The first is the
preganglionic neuron and the second is the postganglionic
neuron. The four possible routes of the preganglionic and
postganglionic fibers, as illustrated in Fig. 14-3, are
summarized below. After entering the sympathetic ganglia via the
white rami, the preganglionic fibers may:
1 Pass
without synapsing up or down the sympathetic chain to ultimately
synapse in a higher or lower ganglion. By passing up the chain,
the first four or five thoracic cord levels contribute all of
the preganglionic fibers to the superior, middle, and inferior
cervical ganglia. Similarly by passing down the chain, the lower
thoracic and upper lumbar cord levels contribute all of the
preganglionic fibers to the ganglia in the chain below L2.
Postganglionic fibers then leave the ganglia via their gray rami
to enter their respective spinal nerves for distribution to
their effector organs (cutaneous and skeletal muscle blood
vessels. sweat glands, and pilomotor smooth muscle).
2 Synapse
in the ganglia and subsequently stimulate postganglionic fibers
which leave the ganglia to reenter the spinal nerves via the
gray rami. The postganglionic fibers are then distributed with
the spinal nerves to their effector organs (cutaneous and
skeletal muscle blood vessels. sweat glands, and pilomotor
smooth muscle).
3 Synapse
in the ganglia and subsequently stimulate postganglionic fibers
which leave the ganglia and are directly distributed to their
effector organs (smooth muscle, visceral organs. blood vessels,
and glands of the head, neck, and thorax).
4 Pass
without synapsing into the abdomen to synapse in one of the
prevertebral ganglia or the adrenal medulla. Postganglionic
fibers leave the prevertebral ganglia to innervate their
effector organs (smooth muscle. visceral organs. blood vessels,
and glands of the abdomen and pelvis).
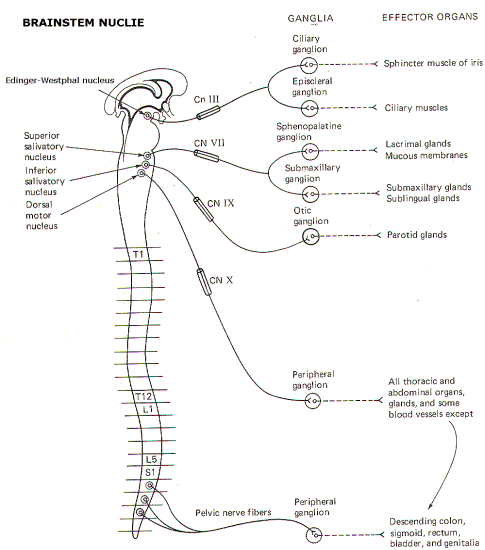 |
THE
PARASYMPATHETIC OUTFLOW
The nerve fibers
which comprise the parasympathetic system originate in two quite
distant regions, the brain stem and the sacral portion of the
spinal cord. For this reason it is often called the
craniosacral outflow to distinguish it from the
thoracolumbar outflow of the sympathetic system. Those GVE
fibers which make up the cranial portion of the system originate
in specific brain stem nuclei and are distributed with cranial
nerves III,
VII,
IX, and X. Those which comprise the sacral portion originate in
lamina VII
of
sacral cord segments 2 to 4 and are distributed as the GVE
fibers of the pelvic nerves (nervi erigentes). As with the
sympathetic system, there are always two neurons in the pathway
to the effector organ supplied. Thus, there are pre- and
postganglionic fibers in the parasympathetic system also.
However, unlike those in the sympathetic system, parasympathetic
ganglia are quite distant from the brain stem and cord, often
located directly on the effector organ itself. Thus the
postganglionic fibers are much shorter in the parasympathetic
system than they are in the sympathetic system. It should be noted
here that autonomic effector organs typically receive both
sympathetic and parasympathetic innervation, though some receive
input from one system only. The effects of sympathetic and
parasympathetic stimulation of the autonomic effector organs are
summarized in Table-1. The effects are often but not always
opposite, as will be described later.
Figure-4
illustrates the parasympathetic outflow. The EdingerWestphal
nucleus (an accessory nucleus of III) in the tegmentum of the
midbrain gives rise to the preganglionic parasympathetic fibers
of the oculomotor (III) nerve. Some of these fibers terminate in
the ciliary ganglion and others in the episcleral ganglion. The
former stimulate postganglionic fibers innervating the sphincter
muscles of the iris, which control pupillary diameter, while the
latter stimulate postganglionic fibers innervating the ciliary
muscle controlling the curvature of the lens (Fig-5).
The superior
salivatory nucleus in the pons gives rise to the preganglionic
fibers of the facial (VII) nerve. Some of these fibers terminate
in the sphenopalatine ganglion and others in the submandibular
(submaxillary) ganglion. Postganglionic fibers from the former
innervate the lacrimal gland and mucous membranes in the head
and neck region while postganglionic fibers from the latter
innervate the submaxillary and sublingual salivary glands. The
inferior salivatory nucleus at the pontomedullary border gives
rise to the preganglionic fibers of the glossopharyngeal nerve
(IX). These fibers terminate in the otic ganglion, from which
postganglionic fibers innervate the parotid gland.
The overwhelming
majority of cranial preganglionic fibers are distributed within
the vagus (X) nerve. They originate in the dorsal motor nucleus
of X in the medulla and terminate in unnamed peripheral ganglia
on thoracic and abdominal organs, glands, and some blood
vessels. Short postganglionic fibers run from these ganglia to
receptor sites on the effector organ cells.
The sacral
parasympathetic outflow supplies the organs and glands in part
of the lower abdomen and all of the pelvis. Included are the
descending colon, sigmoid, rectum, bladder, and external
genitalia. As noted earlier, the preganglionic fibers originate
in lamina VII of the sacral cord between S2 and S4, These fibers
travel with the pelvic nerve and terminate in peripheral ganglia
on the effector organs themselves.
|
Fig-4: The parasympathetic outflow |
|
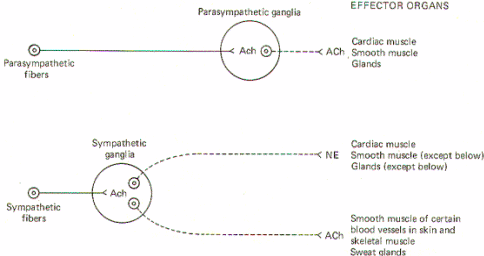 |
AUTONOMIC NEUROTRANSMITTERS
Both sympathetic
and parasympathetic preganglionic neurons are cholinergic;
that is, the preganglionic fibers of both systems release
acetylcholine (ACh) at the synapse in the ganglion. Thus ACh is
the principal transmitter in the autonomic ganglia. There are
also some dopaminergic (dopamine releasing) interneurons
present, but their function is still unknown. Nevertheless, the
preganglionic fibers themselves are all cholinergic.
All postganglionic
fibers of the parasympathetic system are cholinergic, but
postganglionic sympathetic fibers are more diverse. The
overwhelming majority are adrenergic [release
norepinephrine (NE)], but a few are cholinergic. The few which
are known to be cholinergic are those which innervate the sweat
glands and some cutaneous and skeletal muscle blood vessels.
(Fig-5)
|
Fig-5: General scheme of autonomic
neurotransmitters. |
|
Acetylcholine Synthesis,
Release, and Inactivation
Figure-6
illustrates the general scheme of activity at the cholinergic
synapse. Synthesis of ACh occurs in the cytoplasm of
cholinergic presynaptic terminals. Coenzyme A (CoA) combines
with acetate to form acetyl coenzyme A (acetyl CoA). Energy for
this reaction is supplied by ATP. Once formed, the acetyl CoA
combines with choline in the presence of the enzyme choline
acetyltransferase to form acetylcholine (ACh). Once
synthesized, ACh is taken up by the synaptic vesicles and held
there in a bound form until its released.
When an impulse
reaches the presynaptic terminal, several synaptic vesicles
release ACh into the synaptic cleft. ACh then diffuses across
the cleft to activate cholinergic receptor sites on the
postsynaptic membrane. In order to allow the presynaptic
terminal to effectively control the postsynaptic membrane, the
released ACh must be quickly degraded (within microseconds) by
the enzyme acetylcholinesterase (AChE) to acetate and choline,
which are then reabsorbed into the presynaptic terminal for
resynthesis to ACh. A small fraction is reabsorbed intact into
the presynaptic terminal while an even smaller fraction diffuses
out of the synaptic cleft before it can be degraded or
reabsorbed. AChE is abundantly available in the cholinergic
synaptic cleft. And even though the enzyme can degrade ACh
within microseconds, there is adequate time for the ACh to
activate receptor sites.Norepinephrine
Synthesis, Release, and Inactivation.
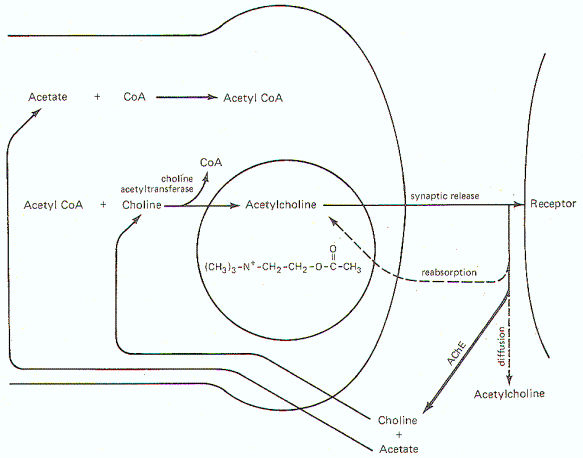 |
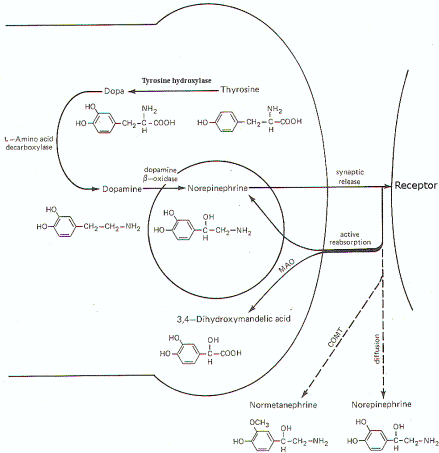 |
Fig-6: Synthesis and fate of
synaptically released acetylcholine at cholinergic
synapse. |
Fig-7: Synthesis and fate of
synaptically released norepinephrine at adrenergic
synapse. |
Figure-7 illustrates the synthesis and fate of synaptically
released norepinephrine at adrenergic synapses. Norepinephrine
is synthesized in the presynaptic terminal by a series of
enzymatically catalyzed reactions typically starting with the
amino acid tyrosine. The sequence can also start with
phenylalanine, which can be enzymatically converted to tyrosine.
In either case tyrosine is converted to dihydroxyphenylalanine (dopa),
dopamine, and finally to norepinephrine. The final synthetic
step from dopamine to norepinephrine occurs in the synaptic
vesicle where the norepinephrine is held in a bound form. The
formation of dopa is apparently the rate-limiting step in the
synthesis of norepinephrine. When an impulse
reaches the presynaptic terminal, several vesicles release norepinephrine into
the synaptic cleft, where it diffuses to activate receptor sites
on the postsynaptic membrane. Within a few milliseconds, the
norepinephrine is subject to one of three fates. A small amount
is methylated by the enzyme
catechol-o-methyl transferase
(COMT), which is
present in the cleft, and thereby rendered inactive. An even
smaller fraction diffuses out of the cleft and away from
receptor sites. But certainly the greatest amount of
norepinephrine is reabsorbed by active transport into the
presynaptic terminal. If norepinephrine stores in the synaptic
vesicles are low, as might be the case in a rapidly firing
fiber, the reabsorbed norepinephrine may be taken up by the
vesicles for subsequent rerelease. If adequate stores of the
transmitter are available, the reabsorbed norepinephrine is
subjected to oxidative deamination by mitochondrial
monoamine oxidase
(MAO).
AUTONOMIC TONE
Table1 shows
the effects of sympathetic and parasympathetic stimulation on
autonomic effector organs. The sympathetic and parasympathetic
systems are continually active and the level of activity at a
given rate of firing is known as
autonomic tone.
Table-1 Autonomic Effects on
Various Organs of the Body
|
Effector organs
|
Effects of sympathetic
stimulation
|
Effects of parasympathetic stimulation |
Eye |
Radial muscle of the iris |
(α)
Contraction (mydriasis) |
|
|
Sphincter muscle of the
iris |
|
Contraction (myosis) |
|
Ciliary muscle of the lens |
(β)
Relaxation Lens flattens |
Contraction ( Lens curves) |
Heart |
SA node |
(β)
↑ heart rate |
↓
heart rate |
|
Atria |
(β)
↑ heart rate and force |
↓
heart force |
|
AV node |
(β)
↑ conduction velocity |
↓
conduction velocity |
|
Purkinje system |
(β)
↑ conduction velocity |
|
|
Ventricles |
(β)
↑ heart rate and force |
|
Blood vessels |
Coronary |
(α)
Constriction |
Dilatation |
|
|
(β)
Dilatation |
|
|
Cutaneous |
(α)
Constriction |
|
|
|
(ACh)
Dilatation |
|
|
Skeletal muscle |
(α)
Constriction |
|
|
|
(β)
Dilatation |
|
|
|
(ACh)
Dilatation |
|
|
Abdominal visceral |
(α)
Constriction |
|
|
|
(β)
Dilatation |
|
|
Renal |
(α)
Constriction |
|
|
Salivary glands |
(α)
Constriction |
Dilatation |
Stomach |
Motility and tone |
(β)
Decrease (usually) |
Increase |
|
Sphincters |
(α)
Contraction (usually) |
Relaxation
(usually) |
|
Secretion |
Inhibition (?) |
Stimulation |
Intestine |
Motility and tone |
(α,
β) Decrease |
Increase |
|
Sphincters |
(α)
Contraction (usually) |
Relaxation
(usually) |
|
Secretion |
Inhibition (?) |
Stimulation |
Gallbladder and ducts |
|
Relaxation |
Contraction |
Urinary bladder |
Detrusor |
(β)
Relaxation (usually) |
Contraction |
|
Trigone and sphincter |
(α)
Contraction |
Relaxation |
Ureter |
Motility and tone |
Increase
(usually) |
Increase
(?) |
Male sex organs |
|
Ejaculation |
Erection |
Skin |
Pilomotor muscles |
(α)
Contraction |
|
|
Sweat glands |
(α)
Slight, localized
secretions |
|
|
|
(ACh)
Generalized secretions |
|
Spleen capsule |
|
(α)
Contraction |
|
Lung (bronchial muscles) |
|
(β)
Relaxation |
Contraction |
Adrenal medulla |
|
|
Secretion of epinephrine
and norepinephrine |
Liver |
|
(β)
Glycogenolysis |
|
Pancreas |
Acinar cells |
↓
secretion |
Secretion |
|
Islet cells |
(α)
Inhibition of insulin
and glucagon secretion |
Insulin and glucagon secretion |
|
|
(β)
Insulin and glucagon
secretion |
|
Salivary glands |
|
(α)
Thick, sparse secretion |
Profuse, watery secretion |
Lacrimal glands |
|
|
Secretion |
Nasopharyngeal glands |
|
|
Secretion |
Adipose tissue |
|
(β)
Lipolysis |
|
Juxtaglomerular cells |
|
(β)
Renin secretion |
|
Pineal gland |
|
(β)
Melatonin synthesis and
secretion |
|
Sympathetic Tone
To illustrate
sympathetic tone, consider this example. Most arteries are
normally in a state of partial constriction. That is, they are
neither fully constricted nor fully dilated. Since most blood
vessels receive only sympathetic innervation, it is the only
system that need be considered. If the normal partially
constricted state of an artery is maintained by a basal firing
rate of 1 impulse per second, we can describe the artery as
displaying a basal sympathetic tone. Now if the firing rate
should increase to say 50 impulses per second, the artery would
constrict further, showing an increase in sympathetic tone.
Conversely, if the firing rate were to decrease, the smooth
muscle of the blood vessel would relax, causing the artery to
vasodilate with a decrease in sympathetic tone.
The adrenal medulla is also an important contributor to
sympathetic tone throughout the body. Each time the sympathetic
system is activated, the adrenal medullae are also sufficiently
stimulated via the splanchnic nerves, to increase their output
of epinephrine and norepinephrine to the general circulation.
These two catecholamines then travel to all parts of the body
stimulating sympathetic effector organs. It is easy to see how
the increased release of these two chemicals by the adrenal
medulla can cause a general increase in sympathetic tone
throughout the body. In fact. this increased output by
the adrenal gland with sympathetic stimulation is the principal
reason why the effects of sympathetic stimulation are longer
lasting and more diffuse than those associated with the
parasympathetic system.
Parasympathetic Tone
An example of
parasympathetic tone is the control of peristalsis in the GI
tract. Gastrointestinal smooth muscle receives both sympathetic
and parasympathetic innervation. Increasing the firing rate of
parasympathetic fibers to the gut causes an increase in
intestinal motility and peristalsis, and hence, an increase in
parasympathetic tone. Decreasing the firing rate produces a
decrease in peristaltic activity, and hence, parasympathetic
tone. Table-1 shows parasympathetic stimulation increases
peristalsis while sympathetic stimulation decreases it. Thus,
the GI musculature is an example of the often true observation
that the effects of sympathetic and parasympathetic stimulation
are opposite and tend to balance each other. Further examination
of Table-1 , however, will show that this is not always
true.
Alpha and Beta Receptors
The action of
catecholamines on adrenergic effector organs varies with the
organs. Catecholamines excite some effectors and inhibit
others. Experiments with a series of sympathetic drugs have
shown there are at least two types of adrenergic receptors. They
are called alpha
and beta. Blocking
agents were later developed for each receptor which further
confirmed their existence. The response of an effector to a
catecholamine is then partly a function of the type of receptor
it has. Epinephrine excites both alpha and beta receptors quite
equally, while norepinephrine excites mainly alpha receptors.
Nevertheless, norepinephrine will also excite beta receptors,
but only to a slight extent. This explains why epinephrine has a
much stronger effect on the heart (which has only beta
receptors) than norepinephrine does. To further confuse the
picture, some effectors have only alpha receptors, others have
only beta receptors, and still others have both. Thus the
specific response of an effector is both a function of the
relative ratio of receptor types and the kind of transmitter
involved. A partial list of the effects of alpha and beta
stimulation is given in Table-2.
Table-2
Effects of Alpha and Beta Stimulation
|
Alpha
receptor |
Beta receptor |
Vasoconstriction |
Vasodilation |
Mydriasis (pupil dilation) |
Cardioacceleration |
Intestinal relaxation |
Bronchial relaxation |
|
Increased cardiac strength |
|
Glycogenolysis |
|
Lipolysis |
Notice that some
alpha functions are inhibitory while others are excitatory. The
same is true for certain beta effects. Therefore it is not
possible to refer to one receptor as excitatory and the other as
inhibitory, as is sometimes true. Beta receptors have also been
divided into two types: beta1 and beta2, according to their
responses to various drugs.
Beta1 receptors
are those
responsible for the inotropic (strength) and chronotopic (rate)
responses of the heart. as well as lipolysis.
Beta2 receptors
bring about
vasodilation and bronchial relaxation. This is a distinction
useful to the pharmacologist, who can then use a
beta2
agonist to treat
asthma and produce bronchial relaxation with very little cardiac
stimulation.
AUTONOMIC AND RELATED DRUGS
A large number of
drugs have been developed which are active at various sites in
the autonomic nervous system. Figure 10 schematically
illustrates the action and site of action of several of these.
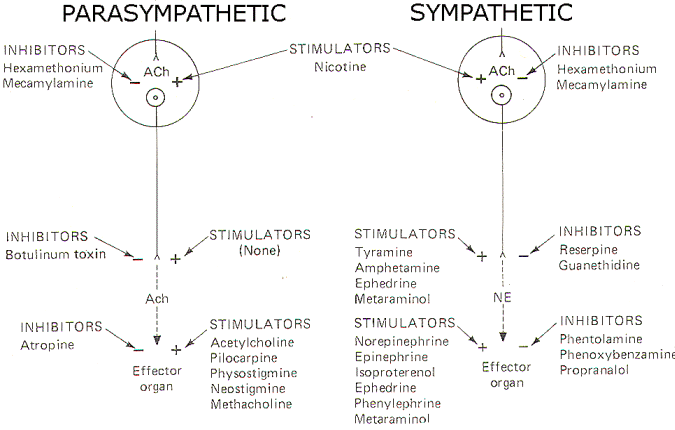
Fig-8: Action of various drugs on the autonomic nervous system.
Drugs Acting on Autonomic
Effector Organs
Acetylcholine,
pilocarpine, and methacholine all directly stimulate cholinergic
receptors on autonomic effector organs. Physostigmine and
neostigmine also potentiate activity at these receptors, but do
it by the indirect action of inhibiting cholinesterase (AChE).
Conversely, atropine is a potent antagonist at these receptors,
inhibiting the action of endogenously released ACh as well as
administered cholinomimetic drugs.
A variety of drugs
are also active at adrenergic receptors on autonomic effector
organs. Norepinephrine, epinephrine, isoproterenol (a beta
agonist) and phenylephrine (an alpha agonist) are all capable of
directly stimulating these receptors. In addition,
ephedrine and metaraminol can act directly on these receptors
but typically are first absorbed by the adrenergic nerve endings
and subsequently released upon the arrival of impulses at the
presynaptic terminal. Metaraminol is an alpha agonist both
directly and indirectly, while ephedrine is a beta agonist
directly but stimulates alpha receptors when released by
adrenergic nerve endings. On the other hand, phentolamine and
phenoxybenzamine are effective alpha antagonists and thus
effectively block alpha receptors. Propranolol is a beta
blocker.
Drugs Acting on Autonomic
Nerve Endings
There are no known
drugs to stimulate the release of ACh from the presynaptic
terminals of cholinergic nerve endings. However, botulinum toxin
is a potent inhibitor of ACh release. Adrenergic nerve endings
are more commonly manipulated by drug action. Both tyramine and
amphetamine promote the release of endogenous norepinephrine
from these nerve endings. Ephedrine and metaraminol are also
potentiators at these sites by the indirect action of being
absorbed into the terminals and subsequently being released as
false transmitters. Reserpine and guanethidine are effective
inhibitors here by the action of depleting stores of norepinephrine in synaptic vesicles and preventing their further uptake and storage.
Drugs Acting on Autonomic
Ganglia
Drugs active at
parasympathetic ganglia are equally effective at sympathetic
ganglia, and vice versa. Nicotine stimulates postganglionic
neuron receptors in the autonomic ganglia. Hexamethonium and
mecamylamine effectively block these "nicotinic"
receptor sites.
Nonautonomic Drugs
It is worth
pointing out that there are several drugs which are active at
the skeletal neuromuscular junction which are not active in the
autonomic nervous system. For example, curare and succinylcholine effectively block the action of ACh on skeletal
muscle receptors but have no similar ACh blocking action on
cardiac, and smooth muscle receptors.
|
 |
 |
Our brain is a mystery and to understand it, you
need to be a neurosurgeon, neuroanatomist and neurophysiologist.
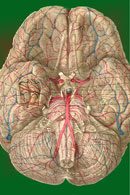
neurosurgery.tv

Please visit this site, where daily neurosurgical activities are going
on.
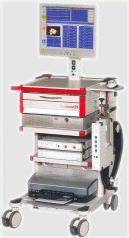
Inomed ISIS IOM System
|
|
|
|