MUSCLE TONE - SPINAL REFELXES
Muscles are
always at least partially contracted. Even seemingly relaxed
muscles possess a small degree of tension called resting muscle
tonus or tone. This tone is ultimately controlled by impulses
from the brain, though special receptors in the muscles
themselves are also instrumental in its regulation. The brain
relies on input from these receptors as well as those in tendons
and joints to give it the information it needs to direct smooth
and coordinated muscle movements. They constantly supply the
brain with necessary information concerning the ever-changing
tone in muscles as well as the present position of muscles at
any time during a movement.
Many
aspects of posture and movement depend on appropriately
controlled and subsequently monitored tone in the large postural
muscles. Here, we will examine how muscle tone is regulated both
by the brain and spinal cord and how the brain is kept informed
of the ever-changing status of this tone. A second objective
will be to examine spinal reflexes. It is easy for the beginner
to treat reflexes lightly, associating them only with visible
activities such as the knee jerk. In fact, the vast majority of
reflex actions are unseen and unnoticed and yet are vitally
important to normal function. Reflexes operating though the
spinal cord are responsible for the smooth functioning of the
gastrointestinal tract and bladder as well as all of the skilled
movements of the trunk and limbs and the often-taken-for-granted
activities of standing erect, walking, and running.
OVERVIEW OF MUSCLE TONE
The muscle
tone exhibited by otherwise relaxed muscles is necessary for
these muscles to produce effective movements. If muscles relaxed
completely (no resting tone), they would overlengthen, and too
much time would be required to take up slack when a contraction
was called for. On the other hand, too much tone would not allow
for sufficient rest and recovery.
The
principal regulator of muscle tone is the small
stretch-sensitive intramuscular unit called the muscle spindle.
Muscle spindles are encapsulated units within the belly of a
muscle that lie parallel to the muscle fibers, stretching when
the muscle is stretched and shortening when the muscle
contracts. Thus they are uniquely situated to detect slight
changes in muscle tone. When stretched, muscle spindles become
activated, causing an increase in the impulse firing rate of
afferent nerve fibers from the spindles to the spinal cord. Some
of these spindle afferents synapse on second-order neurons which
conduct the stretch information up the spinal cord to the
cerebellum and even the cerebral cortex. Since the firing rate
of these neurons varies with the degree and velocity of stretch,
the CNS is continually informed of the ever-changing status of
muscle tone and movement.
Other
spindle afferents directly excite large alpha motor neurons
innervating skeletal muscle fibers. This reflex activation
causes contraction (and shortening) of the muscle via the
simple myotatic or stretch reflex. This reflex functions as a
servo-mechanism to maintain muscle tone at a preset level. If
tone in a particular muscle decreases, allowing the muscle to
lengthen, the spindles become stretched and trigger increased
impulse firing in the spindle afferents, thereby increasing the
firing rate of the alpha motor neurons to that same muscle and
causing it to contract. The stretch sensitivity of the spindles
can be adjusted by action of the small gamma motor neurons in
the anterior horn (lamina IX) of the spinal cord. This is an
important capability, allowing the CNS to keep the spindles "in
tune" with the muscles. These and other functions of the muscle
spindles, as well as the tension-sensitive organs in tendons,
will be discussed.
THE MUSCLE SPINDLE
Anatomy
Muscle
spindles are found in all skeletal muscles. They are more highly
concentrated in muscle utilizing fine delicate control and less
so in the large antigravity support muscles. The greatest
percentage of spindles are located in the belly of the muscle.
Spindles contain two types of intrafusal fibers. Both types are
multinucleated contractile cells (Fig-1).
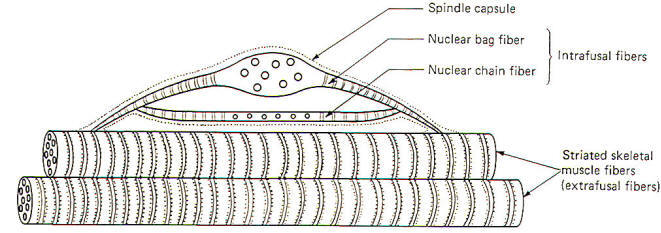 |
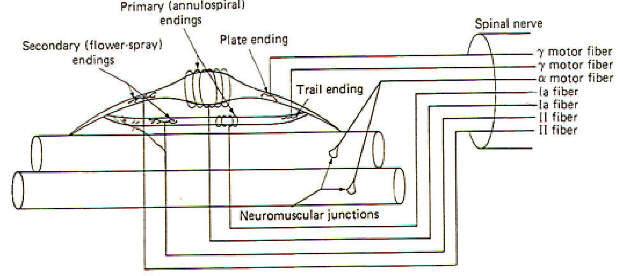 |
Fig-1 |
Fig-2 |
Nuclear bag
fibers receive their name from the fact that their nuclei are
clustered together in a baglike enlargement near the center of
the fiber. Nuclear chain fibers, on the other hand, have no
central enlargement, and their nuclei are spread out in a
chainlike fashion in the equatorial region of the fiber. Both
types are able to contract as contractile myofilaments are
present in their striated peripheral portions. Nuclear bag
fibers typically have greater diameters and are longer than
chain fibers. A typical muscle spindle might contain up to eight
chain and one or two bag fibers. The shorter chain fibers are
often attached to the bag fibers, which in turn attach to the
endomysium of the extrafusal muscle fibers. Extrafusal fibers
are the large contractile fibers of the muscle, while the
intrafusal fibers are the nuclear bag and chain fibers within
the encapsulated muscle spindles.
Innervation of the Spindles
Before
examining the role of the muscle spindle in regulating and
responding to changes in muscle tone. let's first begin by
looking at its neural connections (Fig-2). Each nuclear bag
fiber has both motor and sensory innervation. One or two gamma
motor neurons form several distinct motor end plates, or plate
endings, with the contractile portions of the fiber. Firing of
the gamma fibers contracts and shortens the bag fibers, a
feature which we will see is important in setting the
sensitivity of the spindle. Stretch of the nuclear bag fibers is
detected by specialized stretch-sensitive endings of both group
Ia and group II nerve fibers. The Ia fibers form primary endings
(annulospiral endings) by wrapping around the central region of
the bag fibers. Group II fibers form secondary endings
(flower-spray endings) over the striated portions of the bag
fibers. The nuclear chain fibers also have both motor and
sensory innervation.Very small gamma motor neurons form rather
nondistinct trail endings on the contractile portion of the
chain fibers rather than the more distinct plate endings of bag
fibers. Group Ia and II nerve fibers also form primary and
secondary endings with the chain fibers.
The Myotatic (Stretch) Reflex
When a
muscle is stretched, the spindles in that muscle are also
stretched. Stretch of the nuclear bag and chain fibers in the
spindles stimulates the primary and secondary endings of the Ia
and II afferent fibers, causing them to send impulses into the
cord. Many of these fibers (particularly the Ia fibers) synapse
directly on alpha motor neurons supplying the same muscle which
was initially stretched. This causes the muscle to contract and
shorten, relieving the initial stretch. Such neurons are called
homonymous alpha motor neurons. This
"stretch-resulting-in-relieved-stretch" is known as the myotatic
or stretch reflex. Once the muscle contracts and the stretch is
relieved, the firing rate of the spindle afferents returns to
the resting level (Fig-3).
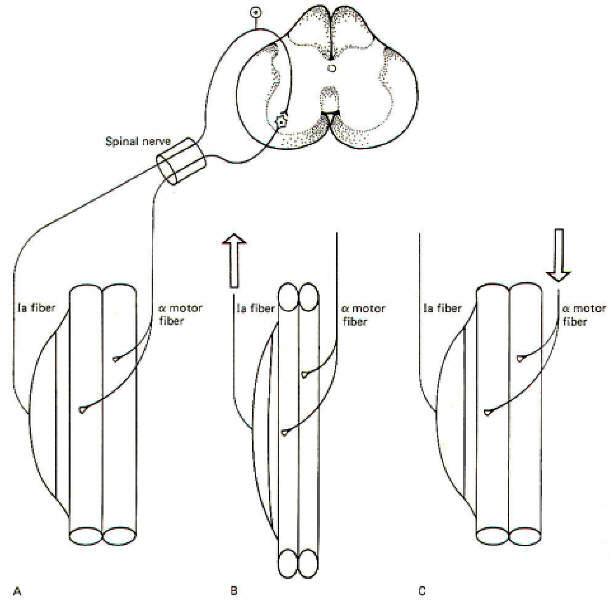 |
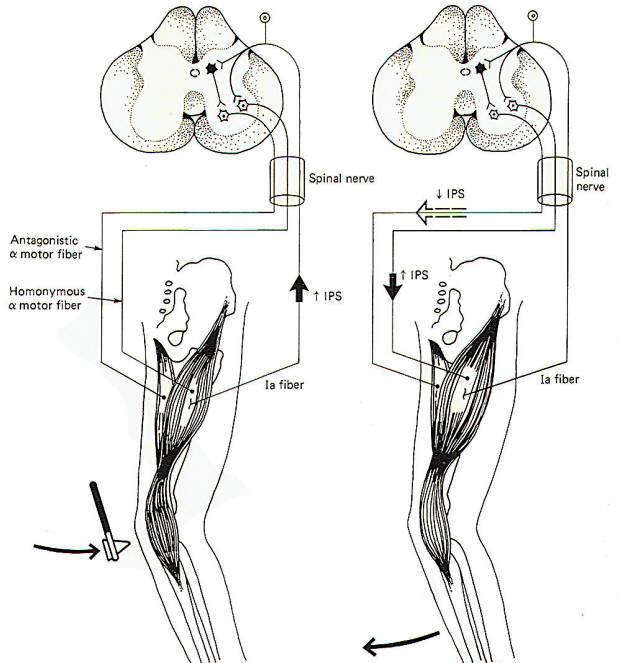 |
Fig-3 |
Fig-4 |
Skeletal
muscles are attached to the skeleton in order to bring about
movements of the body. It is usually necessary for muscles
opposing a reflex movement (antagonists) to relax while those
producing the movement (agonists) contract. This reciprocal
action requires the incorporation of inhibitory interneurons in
the spinal cord. Branches (collaterals), typically from the Ia
spindle afferents, synapse in the posterior horn of the spinal
cord gray matter. Here they stimulate inhibitory interneurons
which depress activity in the alpha motor neurons to those
muscles antagonistic to the desired movement. The patellar
tendon or knee jerk reflex illustrates this point in Fig-4.
When the
tendon is tapped with a reflex hammer, the anterior thigh
(quadriceps) muscles and many of its muscle spindles are
stretched. Accordingly, volleys of impulses are sent into the
spinal cord over the spindle afferents. Those fibers synapsing
directly on homonymous alpha motor neurons bring about
contraction of the quadriceps, causing the leg to kick in the
classic response. Of course the posterior thigh muscles
(hamstrings) must relax in order to allow this to happen. This
is accomplished by spindle afferent stimulation of inhibitory
interneurons (Renshaw cells). Once activated, they depress
firing in the alpha motor neurons to the antagonistic muscles.
Renshaw cells release the inhibitory neurotransmitter GABA at
their synapses. Notice that the same spindle afferents which
increase the firing rate in the homonymous alpha motor neurons
decrease activity in the antagonistic motor neurons. The latter
is accomplished through "feed-forward" inhibition. Keep in mind
that the spindle afferents are excitatory neurons releasing ACh
at their synapses. The desired inhibition of the antagonistic
alpha motor neurons is "fed forward" through the inhibitory
interneuron, the Renshaw cell.
The Gamma Efferents and Spindle
Sensitivity
Up to this
point we have only been concerned with the action of the muscle
spindle afferents on alpha motor neurons. Now let's examine how
the sensitivity of the spindles can be adjusted to maintain a
preset level of muscle tone. Recall that the spindle afferents
are stimulated whenever the intrafusal fibers are stretched
taut. Now if the intrafusal fibers are already partially
contracted, only a slight amount of stretch is needed to pull
them taut, increasing the firing rate of the spindle afferents.
On the other hand, if the intrafusal fibers are relaxed and
slack, a considerably greater stretch of the muscle is needed in
order to pull them taut and fire the spindle afferents. In other
words, the muscle spindle is more sensitive to stretch when its
intrafusal fibers are partially contracted then when they are
not. The degree of contraction of the intrafusal fibers and thus
the sensitivity of the muscle spindle is controlled by the
activity of the gamma motor neurons. The greater the firing rate
of the gamma efferents, the greater the degree of intrafusal
contraction, and the greater the sensitivity of the spindle.
Spindle Maintenance of a Preset Muscle
Tone
Recognize
that when muscles isotonically contract they shorten. Similarly,
relaxation causes them to lengthen. Now let's assume that a
given muscle is set to maintain a certain degree of contraction
or tone. If the muscle relaxed too much it would lengthen and
its spindles would stretch, initiating the stretch reflex. This
would cause the muscle to contract, thereby relieving the
stretch brought on by the initial relaxation. Similarly, if the
muscle contracted too much, it would shorten and its spindles
would become increasingly slack. This would decrease the
stimulation of the spindle afferents, thereby decreasing the
stimulation of the homonymous alpha motor neurons and causing
the muscle to partially relax. As a result of this
"servomechanical" nature of the muscle spindles, muscle tone
remains very constant at any preset level. Increases in tension
are reflexly countered by relaxation, while decreases in tension
are countered by contraction.
It is
important to recognize that tone is regulated by the stretch
reflex and is not a characteristic of the muscle itself. This
can be demonstrated by the immediate loss of muscle tone which
occurs when the reflex arc is interrupted at any point. For
example, sectioning either the anterior or posterior roots of
spinal nerves results in the immediate loss of tone to all those
muscles involved.
"Tuning" the Muscle Spindles
In order to
remain sensitive to the slightest change in muscle tone it is
important that the spindles not be allowed to go completely
slack. Under normal conditions intrafusal spindle fibers are
partially contracted. In this state, a slight relaxation or
stretch of the muscle will be detected by the spindles as will a
slight contraction or shortening. The firing rate of the spindle
afferents will increase or decrease accordingly, and the
spindles are said to be "in tune" with the muscle.
One of the
important roles of muscle spindles is to keep the brain and
particularly the cerebellum continually informed of even slight
changes in muscle tone. This is accomplished via collaterals
from the spindle afferents which synapse on neurons of the
spinocerebellar tracts. The second-order neurons of these tracts
conduct information concerning the state of muscle tone and
movement to this important coordinating center of the brain
(Fig-7). Now consider what would happen if the motor cortex of
the brain directed a particular muscle to maintain a higher
level of contraction (tension). Without a simultaneous
contraction of the spindle intrafusal fibers in that muscle, the
spindles would go slack and the firing rate of the spindle
afferents would drop off to zero, producing a "silent period."
Consequently, the spindles would no longer be able to detect
slight increases or decreases in muscle tone and they would be
"out of tune" with the muscle (Fig-5). If, as neurophysiologists
suspect, detecting slight changes in muscle tone is an important
feature of muscle spindles. these would no longer be
contributing, and the cerebellum would be out of touch with
tension changes in the muscle. Fortunately, activity in the
gamma efferent nerve fibers prevent this from happening by
increasing the degree of intrafusal fiber contraction at
approximately the same time that the alpha motor neurons
contract the extrafusal fibers. By this "coactivation" of alpha
and gamma motor neurons, spindles are kept "in tune" with their
muscles (Fig-6).
The role of
the gamma efferents in adjusting the sensitivity of the muscle
spindles has already been discussed. The basal rate of firing of
the gamma efferents and, through them, the contractile state and
sensitivity of the spindles are regulated by the brain through
pathways descending in the spinal cord. The principal route is
the medial reticulospinal tract. This tract, which originates in
the reticular formation of the brainstem, receives input from
many areas of the brain, including the cerebral and cerebellar
cortexes.
Cerebellar "Awareness" of Muscle Tone
The
cerebellum is an important center for the central coordination
of muscle activity. As such, it is necessary for the cerebellum
to be continually informed of progressing body movements and
changes in muscle tone. As previously mentioned, this is
accomplished by collaterals from the spindle afferents which
synapse in the nucleus dorsalis of the spinal cord. Some of the
second-order nerve fibers from this nucleus ascend the cord in
the posterior spinocerebellar tract (PSCT) to enter the
cerebellum via the interior cerebellar peduncle on the same
(ipsilateral) side of the body as the entering spindle
afferents. They terminate in the cerebellar cortex of the vermis
(Fig-7). Other second-order nerve fibers from the nucleus
dorsalis cross over to the opposite (contralateral) side of the
spinal cord and ascend to the brainstem in the anterior
spinocerebellar tract (ASCT), where they cross back to enter the
cerebellum via the superior cerebellar peduncle and terminate in
the vermal cortex.
By "tapping
off " the signals from the spindle afferents and conducting them
cranially over these pathways, the cerebellum is continually
kept informed of the ever-changing status of muscle tone.
Electrophysiological studies indicate that group II fibers
appear to be concerned with relaying information concerning
changes in muscle length, while Ia fibers are concerned with
changes both in length and contraction velocity.
It is
important to recognize that the cerebellum functions as a
coordinator examining the performance of a muscle during a given
movement and comparing it with the intended movement directed by
the cerebral cortex. If the intended performance and the actual
performance don't match up exactly, the cerebellum can take
corrective action to synchronize them through its own output to
the motor system. Therefore it is important for the cerebellum
to continually receive input from the muscle spindles on the
progression of any given movement. Input from Golgi tendon
organs and joint receptors is also necessary for movement
coordination.
THE GOLGI TENDON ORGAN
The tendons
of skeletal muscle contain special receptors called Golgi tendon
organs. These receptors are sensitive to the changes in tension
generated by muscles as they contract. Little is known about
their structure except that they are in intimate contact with
the peripheral endings of group Ib afferent fibers. It is
through impulses generated in these afferent fibers that changes
in muscle tension detected by the tendon organs are relayed to
the spinal cord and brain. As muscles contract and tension is
applied to their tendons, the tendon organs are stimulated,
which in turn propagate impulses over group Ib fibers into the
cord, where they take several divergent routes (Fig-8).
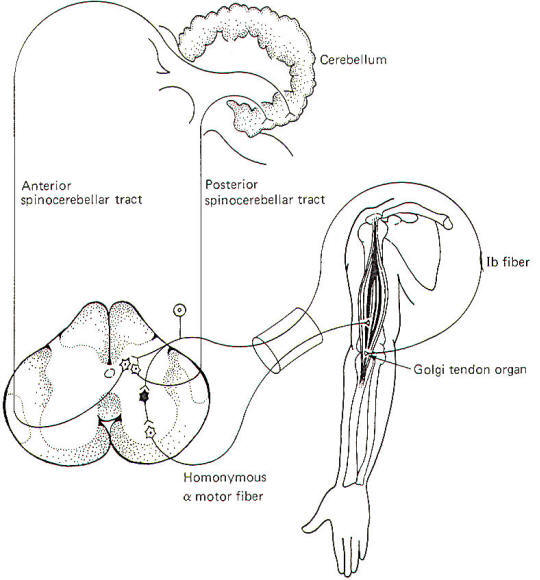 |
Fig-8 |
Function of the Golgi Tendon Organ
The
sensitivity of the tendon organs is considerably less than that
of the muscle spindles. As little as 1 or 2 g of tension is
sufficient to increase the firing rate of the spindle afferents.
On the other hand, the group Ib afferent fibers from the tendon
organs don't register impulse conduction until the tension
reaches as high as 100 g. When tension in the tendons begins to
exceed this level, the tendon organs become sufficiently
stimulated to produce impulse firing in the group Ib fibers.
Like the spindle afferents, the group Ib fibers send collaterals
into the nucleus dorsalis of lamina VII of the spinal cord gray
matter. Subsequently, both ASCT and PSCT second-order neurons
conduct information from the tendon organs to the cerebellum.
If the
tension developed in a strongly contracting muscle becomes
excessive, it is not inconceivable that the tendon could pull
free from the bone, certainly an undesirable situation. However,
before this can happen the tendon organs become sufficiently
stimulated to send large volleys of impulses into the cord to
directly stimulate the alpha motor neurons to antagonistic
muscles and inhibitory interneurons to homonymous alpha motor
neurons. The resulting feed-forward inhibition to the strongly
contracting muscle causes it to suddenly relax, relieving the
strain on the tendon and preventing possible damage. This sudden
relaxation of a muscle in the face of dangerously high tension
is called the lengthening reaction or the "clasp-knife" reflex
because of its similarity to the way a pocketknife suddenly
snaps closed when the blade is moved to a certain critical
position.
It was
originally thought that little if any information from the
tendon organs or the muscle spindles reached the conscious level
in humans. The vast majority of the signals from these receptors
which ascend the cord were thought to be directed exclusively to
the cerebellum for subconscious evaluation. However, recent
evidence now indicates that input from muscle spindles, tendon
organs, and joint receptors is also relayed to the cerebral
cortex and is probably responsible for the conscious sensation
associated with the position and movement of limbs.
OVERVIEW OF SPINAL REFLEXES
A reflex
can be defined as a specific response to an adequate sensory
stimulus. Strictly speaking, this response most often involves a
muscular contraction or a glandular secretion. The spinal
reflexes we will examine here all involve muscular contractions.
A reflex arc is the neural circuit over which the reflex
operates (Fig-9).
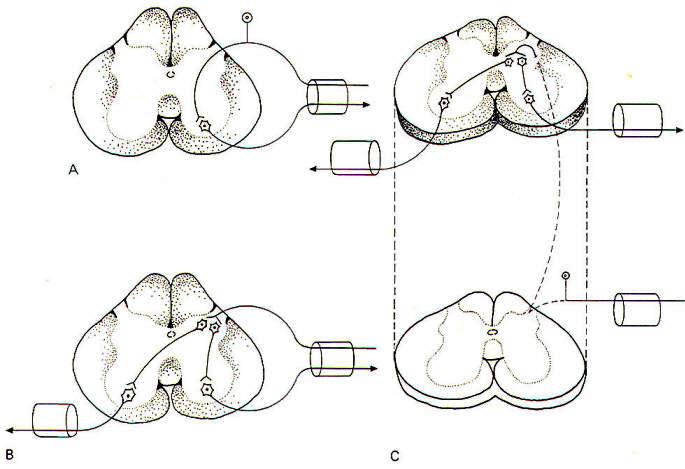 |
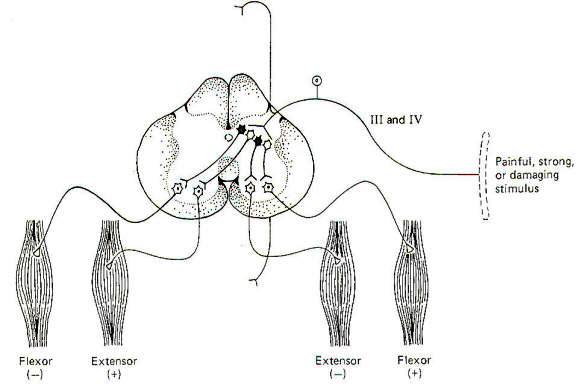 |
Fig-9 |
Fig-10 |
In its
simplest form it involves an afferent neuron conducting impulses
from the point of stimulation into the spinal cord and an
efferent neuron conducting impulses out to an efferent muscle or
group of muscles. This is a monosynaptic or simple reflex
because it utilizes only two neurons and one synapse. If one or
more interneurons in the cord link the afferent and efferent
fibers, the reflex is polysynaptic. If the afferent and efferent
fibers occupy one or just a few cord segments the reflex is
segmental. Intersegmental reflexes involve several cord
segments. If centers in the brain are included in the reflex
pathway. the reflex is supraspinal.
We noted
earlier that it is easy to underestimate the importance of
reflexes. For example, one tends to think of a simple act such
as setting a dinner plate on the table as a purely voluntary act
directed exclusively by the conscious motor cortex of the brain.
In fact, however, the successful completion of this simple task
requires the additional input of polysynaptic reflexes of the
segmental, intersegmental, and supraspinal types. Most of the
neural circuits making up such reflexes are very complex and
poorly understood. Nevertheless, they undoubtedly involve
special application of certain basic reflex types such as the
stretch reflex and others. Let's look at an example of a
somewhat complex spinal reflex which is at least partially
understood.
The Flexor-Crossed-Extensor Reflex
A strong,
painful, or potentially damaging stimulus delivered to cutaneous
or joint receptors can reflexly cause a sudden bodily withdrawal
away from the stimulus. Stepping on a tack is a good example of
this reflex in action. The person will typically flex (withdraw)
the stimulated foot and leg while extending the other leg in
order to propel the body away from the tack. This is a
polysynaptic, bilateral reflex incorporating both excitatory and
inhibitory interneurons. Delivery of the stimulus to the
receptors in a limb increases the firing rate of pain-carrying
group III and IV afferents into the posterior horn. where they
synapse with interneurons (Fig-10). Excitatory interneurons
ipsilaterally stimulate alpha motor neurons to the flexors in
that limb while contralaterally stimulating extenders in the
opposite limb - thus the term flexor-crossed-extensor reflex. At
the same time, inhibitory interneurons ipsilaterally inhibit
extenders of the stimulated limb while contralaterally
inhibiting flexors of the opposite limb.
This reflex
is often intersegmental. This should not be surprising when one
considers that many muscles are involved in such movements. In
the cat, for example, a painful stimulus delivered to one hind
leg will not only reflexly withdraw that leg, but will extend to
both hind legs and forelegs on the opposite side as well. This
means that the group III and IV afferents not only stimulated
interneurons at the same segmental level at which they entered
the cord, but activated synapses at higher and lower cord levels
as well. The ascending and descending collaterals travel in the
fasciculus proprius (ground bundles) of the white matter. The
fibers in these tracts carry intersegmental connections.
ELECTROPHYSIOLOGY OF
SPINAL REFLEXES
Neuronal
synaptic connections in the spinal cord are difficult to examine
experimentally because of their great density and complexity.
The peripheral fibers of a reflex are much easier to study.
Consequently, some knowledge concerning synaptic activity in the
cord can be obtained by electrically stimulating afferent fibers
while recording from synaptically stimulated efferent fibers.
Monosynaptic and Polysynaptic Reflexes
When
afferent nerve fibers in the posterior root are repetitively
stimulated by an electronic stimulator, compound action
potentials can be recorded from anterior root fibers (Fig-11).
The afferent nerve fibers stimulate anterior root neurons either
directly or indirectly. which then conduct recordable impulses
out their efferent fibers. A compound action potential is the
sum of several individual action potentials. It is obtained when
action potentials from several nerve fibers are recorded
simultaneously with the same recording electrodes.
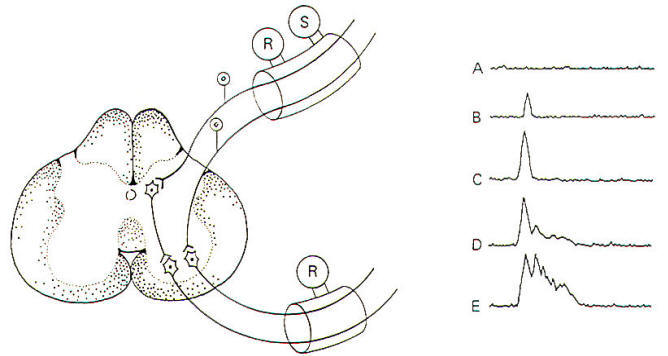 |
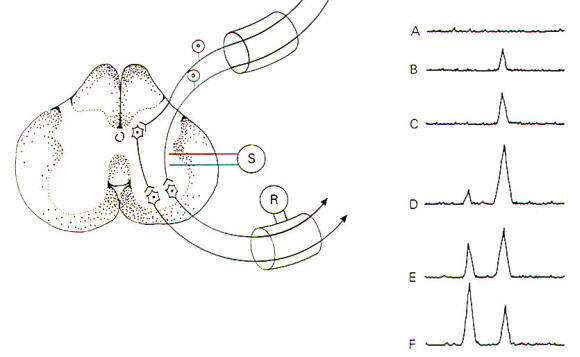 |
Fig-11 |
Fig-12 |
Notice that
when the stimulus is small, the compound action potential is
also small. With increases in stimulus strength, more posterior
root neurons and hence more anterior root neurons are excited
and the size of the action potential increases. With yet further
increases in stimulus strength, two observations can be made.
First, there is again an increase in the size of the compound
action potential as more neurons are recruited, and secondly we
see the appearance of slightly delayed potentials. These latter
potentials are due to polysynaptic relays. Because of the delay
caused by the additional synapses. the resultant impulses reach
the recording electrodes later than the monosynaptic relays.
These polysynaptic responses do not appear if the stimulus
strength is too low because of the failure to sufficiently
stimulate the interneurons. The more interneurons involved, the
stronger the initial stimulus needs to be in order to maintain
excitability through the multiple synapses. As the stimulus
strength is still further increased, relays involving even
greater numbers of synapses are recruited. Finally, when the
posterior root neurons are maximally stimulated, the response
will level off and further increases in stimulus strength will
not change the magnitude of the response.
Determination of Synaptic Delay Time
When the
stimulating electrodes are placed into the lateral region of the
spinal cord itself, stimulation directly excites both afferent
neurons and interneurons (Fig-12). By painstaking and careful
placement of these stimulating electrodes, only one synapse
separates the afferent neurons and interneurons from the
efferent neurons of the anterior horn. As the stimulating
current is increased, both afferent neurons and interneurons
will be sufficiently stimulated to conduct impulses to their
synapses and excite the alpha motor neurons so that compound
action potentials are recorded in the anterior root. As the
stimulus strength increases, more and more afferents and
interneurons are stimulated and the size of the compound action
potential is observed to increase also. With still further
increases in stimulus strength, some of the anterior motor
neurons are stimulated directly by the electrode current spread
through the cord. Since no synapses are involved in this
instance, an earlier compound action potential is also recorded.
The difference in time delay between the appearance of these two
action potentials represents the synaptic delay. Values of 0.5
ms are typical in this kind of experiment. The delay represents
the time it takes for Ca2+ ions to enter the presynaptic
terminal and bring about the subsequent release of
neurotransmitter, followed by diffusion across the cleft and
activation of receptor sites on the postsynaptic membrane. Still
further increases in the stimulus strength produce an increase
in the amplitude of the first potential and a decrease in the
amplitude of the second potential because of the interneurons
finding the motor neurons in a refractory state.
Facilitation and Occlusion in a
Neuronal Pool
Nerve cell
axons often branch into hundreds and even thousands of neuronal
filaments before synapsing with other neurons. As many as 100
neurons are often supplied by a single axon in this manner. Some
of these postsynaptic neurons receive many synaptic inputs from
a single presynaptic neuron while others receive only a few. All
of the nerve cells which receive synaptic input from a single
presynaptic neuron make up the neuronal pool of that neuron.
When a neuron supplying a neuronal pool is firing impulses
repetitively. some of the neurons in the pool are sufficiently
stimulated to establish EPSPs of threshold level, while others
(those receiving few synaptic inputs from the neuron) are not.
Those stimulated to threshold level are in the liminal or
discharge zone of the pool, while the others are in the
subliminal or facilitation zone (Fig-13).
Neuron
pools overlap. That is, some of the neurons in the neuronal pool
of one input neuron are likely to be included in the neuronal
pool of a second and even a third and fourth input neuron. While
the neurons in the facilitation zone of one input neuron are not
sufficiently stimulated to reach threshold by the action of that
neuron alone, they may be raised to the excitation threshold and
begin to fire impulses if they are also in the facilitation zone
of a second simultaneously firing input neuron (Fig-14). This
phenomenon is called facilitation. Facilitation in this case
means that the postsynaptic output from a neuronal pool evoked
by the simultaneous firing of two input neurons is greater than
the sum of each fired separately. When the discharge zones of
two neuronal pools overlap, the opposite effect is observed. In
this case the postsynaptic output from a neuronal pool evoked by
the simultaneous firing of two input neurons is less than the
sum of each fired separately (Fig-15). This is called occlusion.
Convergence and Divergence
Convergence
and divergence are important means by which the central nervous
system channels and sorts different information. There are many
examples of each throughout the nervous system. Synaptic input
to the large alpha motor neuron in the spinal cord anterior horn
is a good example of convergence (Fig-16). We see that several
nerve fibers converge on the motor neuron. each exerting some
measure of influence over the central state of this cell. The
primary sources are probably the corticospinal tract fibers from
the brain. However, we also know that it receives input from the
spindle afferents, group Ib fibers from Golgi tendon organs,
Renshaw cells, and several other pathways descending in the
spinal cord. Because of this funneling of input.
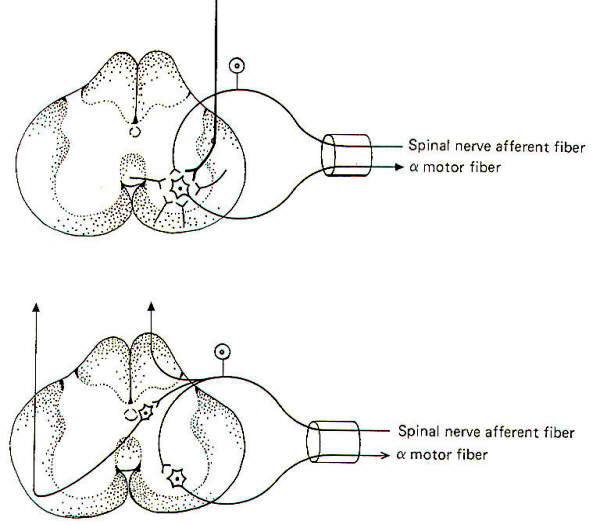 |
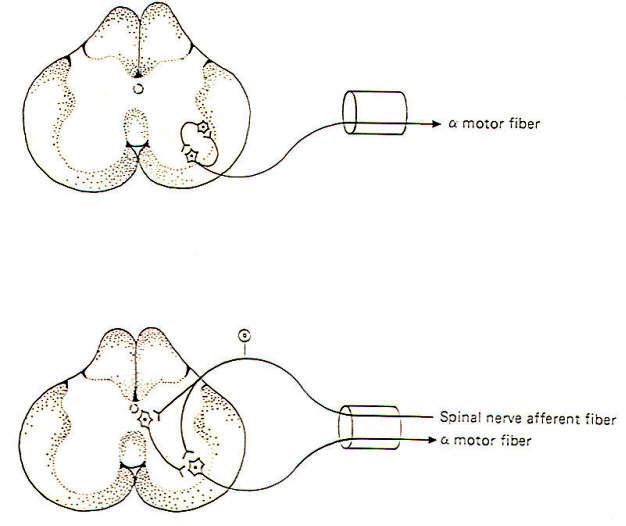 |
Fig-16 |
Fig-17 |
Sherrington
has called the motor neuron the final common pathway in motor
output. Remember that the firing rate of a neuron depends on the
level of its central excitatory state (CES). The higher the CES
in excess of the excitation threshold. the higher the firing
rate. Of course if the CES is less than the excitation
threshold, the motor neuron will not fire at all.
It is often
important that information arising in one area of the body be
transmitted to several different regions in the nervous system.
This spread of information is accomplished by the process of
divergence, Figure 4-16 illustrates the divergence of signals
entering the spinal cord via a spinal afferent fiber which
diverges and takes three separate routes. Two of these are
directed cranially via ascending pathways in the spinal cord.
while the third is routed to a spinal reflex. In another
respect, the transmission of impulses from a single input neuron
to the various neurons in its neuronal pool is also divergence.
Parallel and Recurrent Circuits
It is easy
to picture neurons lined up in single file with the first
stimulating the second and so on. In nature, however, neural
pathways are typically more complex. Two exceptions to the
single-file concept are illustrated in Fig-17. In a parallel
circuit, an incoming neuron stimulates a second neuron both
directly and indirectly (via one or more interneurons). Consider
a neuron (A) which directly excites a neuron (B) through an
excitatory synapse. In addition, neuron A stimulates an
interneuron (C), which in turn excites neuron B. It should be
apparent that if neuron A is stimulated, recording electrodes
placed on neuron B will register two spikes. The first is caused
by neuron A directly stimulating neuron B, and the second is
caused by the delay through the interneuron C synapse. The delay
of this afterdischarge (second spike) is determined by the
number of interneurons involved in the parallel circuit. The
interneurons may be excitatory or inhibitory. When a collateral
branch of a neuron synapses with an interneuron which then
returns to resynapse with itself, either directly or indirectly,
a recurrent circuit is formed. Like parallel circuits, recurrent
circuits may be either excitatory or inhibitory.
|