NEUROEMBRIOLOGY
So many
aspects of human neuroanatomy and neurophysiology refer to early
development that even casually interested one sooner or later
find out they must come to grips with the underlying embryology.
Here, the task is: (1) to present only those neuroembryological
concepts that are necessary for the study of neurophysiology and
(2) to review the major structures and systems involved in
nervous system organization and classification. It is not
intended to be an exhaustive (or exhausting) treatment of the
field.
The basic
units of nervous tissue are its cells. These include neurons,
which are the excitable impulse-conducting cells, as well as
several types of nonexcitable cells. The latter include the
neuroglial and ependymal cells of the central nervous system
(CNS) and the Schwann cells of the peripheral nervous system
(PNS).
A PRELIMINARY OVERVIEW OF NEUROEMBRYOLOGY
Many
neurophysiologists find themselves somewhat baffled by
terminology and classification systems. Many of the terms and
systems are based on embryological origins, and the beginner who
has avoided or not been exposed to embryology will encounter
some difficulty. One major effort here is to present just enough
embryology to explain the origins of the various components of
nervous tissue. The beginner should also come to appreciate the
reasons behind many of the classification schemes for nerve
fibers. receptors, and the various functional divisions of the
nervous system.
The
developing embryo is characterized by three germ layers, which
give rise to the various specialized systems of the body. These
are the ectoderm, the mesoderm, and the entoderm (Fig-1). The
ectoderm is of particular interest to the neuroscientist because
it gives rise to the nervous system as well as the epidermis
with all of its sensory receptors. The mesoderm gives rise to
connective tissue as well as the skeletal, muscular.
circulatory, and urogenital systems and glands. From the
entoderm arise the digestive and respiratory epithelia. The
three germ layers and the tissues which develop from them are
summarized in Fig-2.
About the
seventeenth day following fertilization, the notochord induces
the ectoderm in the middorsal region to differentiate. That is,
it thickens, forms a neural groove, and becomes the neural
ectoderm. During the next 4 days the neural ectoderm begins to
separate from the remaining ectoderm (called the surface
ectoderm) and begins to invaginate, converting the neural groove
to a neural tube with two parallel-running neural crests. This
process is called neurolation
(Fig-3). Soon after formation of the neural tube, the neural
crests subdivide into primordial ganglia. Each of these ganglia
corresponds to a primitive somite or body segment.
Structures
of Neural Crest Origin
The neural
crest gives rise to neuroblasts (cells which later become
neurons) and nonneuroblastic cells which later become the
Schwann cells of the peripheral nervous system. Neuroblasts
eventually give rise to the autonomic postganglionic neurons,
the adrenal medulla, and the afferent neurons of the cranial and
spinal ganglia (Fig-4).
Structures
of Neural Tube Origin
The neural
tube becomes the brain and spinal cord of the central nervous
system. The neuroepithelial cell lining of the neural tube
gives rise to neuroblasts from which arise all CNS neurons with
the exception of those in the mesencephalic nucleus of cranial
nerve V, which are probably of neural crest origin. Certainly
the motor neurons of the cranial, spinal, and preganglionic
autonomic nerves arise from neuroblasts of neural tube origin.
The
nonexcitable cells of the central nervous system also arise from
the neuroepithelial cell lining of the neural tube. Some of
these cells remain fixed in position and become the single layer
of epithelial cells which later line the ventricles of the
brain and the central canal of the spinal cord. This lining is
the ependyma and it separates the cerebrospinal fluid (CSF) of
the ventricles and central canal from the excitable tissue of
the brain and spinal cord. Other cells (glioblasts) migrate
through the neural tube and give rise to the neuroglial cells of
the CNS. The structures which arise from neural tube origin are
illustrated in Fig-5.
Development
of the Brain
The
cephalic end of the neural tube, which is larger than the
posterior end from the very beginning of its embryological
development, gives rise to the brain. The brain includes the
cerebral hemispheres, the brainstem, and the cerebellum. The
embryo, which is essentially a disk during its early stage,
begins to undergo flexion, a process of curving during which
time it is transformed into a tube (Fig-6). It is eventually
joined to its extraembryonic membranes only by a thin stalk, the
umbilical cord. Flexion results from the rapid growth of the
dorsal aspects of the embryo and is characterized by a
relatively rapid growth and development of its cephalic (front)
end into the brain.
The
notochord, a midline structure, is apparently responsible for
inducing the development of the brain and spinal cord. The
notochord itself is mesodermal tissue and eventually gives rise
to the vertebral column and cranium, which enclose and protect
the brain and spinal cord.
Examination
of the 28-day embryo shows that the lumen of the neural tube is
large in the cephalic end and relatively narrow in the rest of
the tube. The large cephalic lumen becomes the ventricular
system of the brain, while the remaining narrow lumen becomes
the central canal of the spinal cord. The ventricular system
and the central canal are continuous with each other and are
filled with CSF. The cephalic neural tube itself becomes brain
tissue proper.
Early on,
three easily identifiable enlargements can be seen in the
embryonic brain. These are the prosencephalon (forebrain), the
mesencephalon (midbrain), and the rhombencephalon (hindbrain).
These are illustrated in Fig-7. The neural tube is initially
open at the anterior pole of the prosencephalon (anterior
neuropore) and also at the posterior end of the neural tube
(posterior neuropore). These pores later close.
With
subsequent development, the prosencephalon differentiates into
the telencephalon (endbrain), which is entirely represented by
the two cerebral hemispheres, and the diencephalon
(betweenbrain), composed of the thalamus, hypothalamus,
subthalamus, and epithalamus. The mesencephalon also continues
to develop but it undergoes no further subdivision. The rhombencephalon gives rise to the metencephalon (afterbrain),
which includes the pons and cerebellum, as well as the
myelencephalon (marrowbrain), which becomes the medulla
oblongata (Fig-8).
|
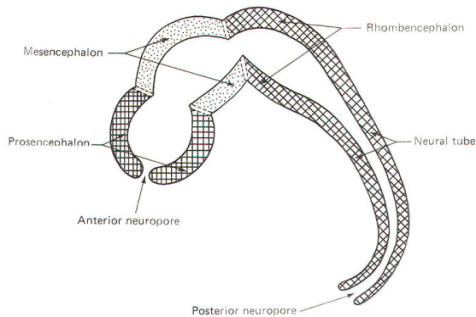 |
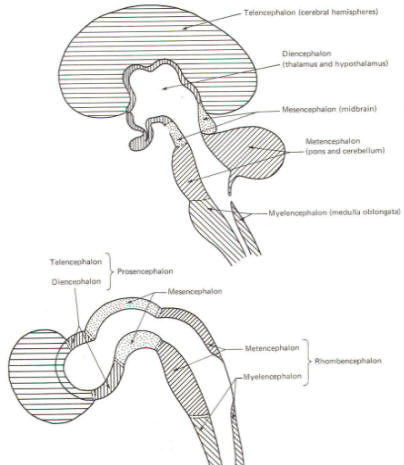 |
|
|
|
Fig-7 |
Fig-8 |
|
|
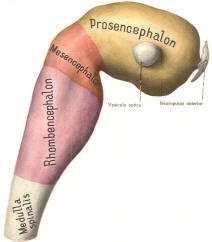 |
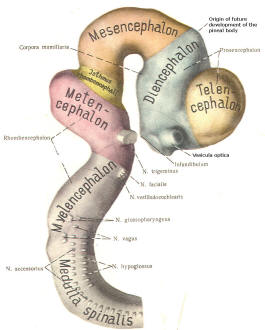 |
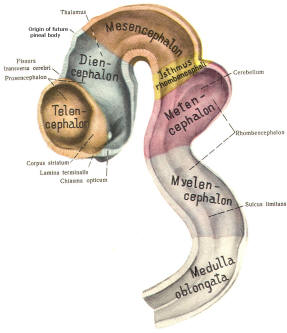 |
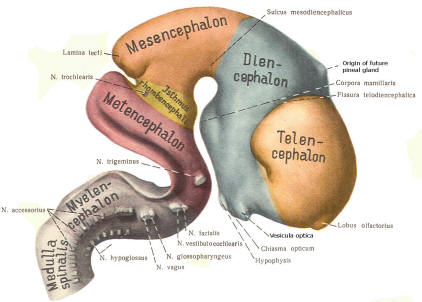 |
Fig-9 |
Fig-10 |
Fig-11 |
Fig-12 |
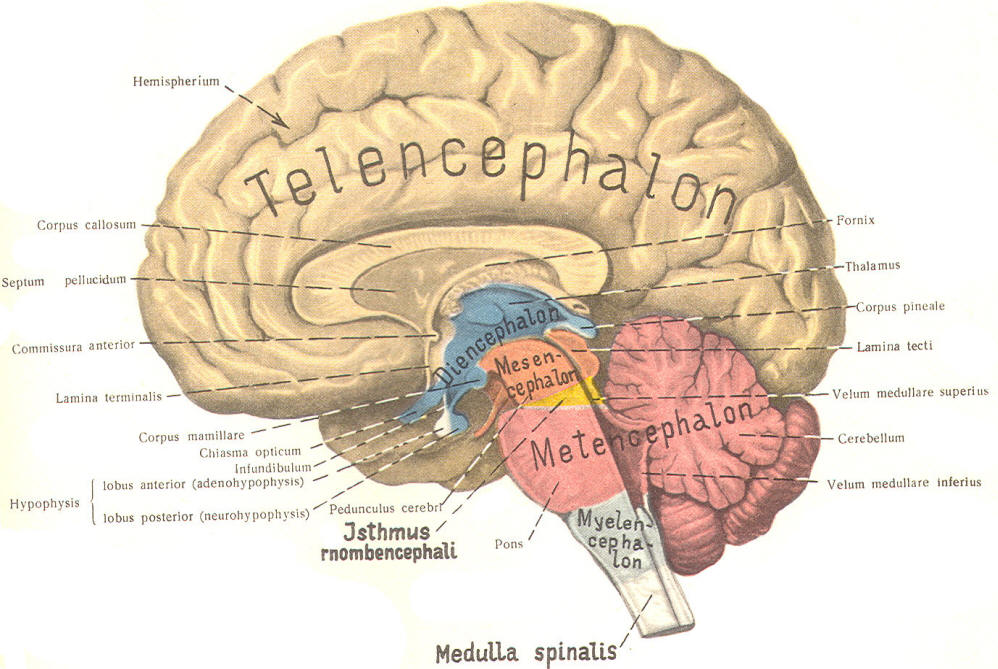 |
Fig-16 |
Meningeal Coverings of the Brain and Spinal
Cord
As the
embryo develops, coverings called the meninges (of mesodermal
origin) develop along with it and completely enclose the brain
and spinal cord. The meninges separate the brain and spinal
cord from the bony surface lining of the cranium and vertebral
canal. Soft meninges or leptomeninges include the piamater, an
extremely thin membrane which is in direct and intimate contact
with the brain and spinal cord, and the arachnoid membrane,
connected to the pia by strands or webs. The dura mater (hard
meninges) is a tough covering which separates the soft meninges
from the bony cranial vault and the vertebral canal. The
leptomeninges may be derived from the neural crest, while the
hard meninges develop from ordinary mesenchyme. The meninges
are illustrated in Fig-17 and 18. In addition to providing protection
to the brain and spinal cord, the meninges serve to accompany
blood vessels to and from CNS tissue as well as to channel CSF
around the exterior surfaces of the brain and spinal cord.
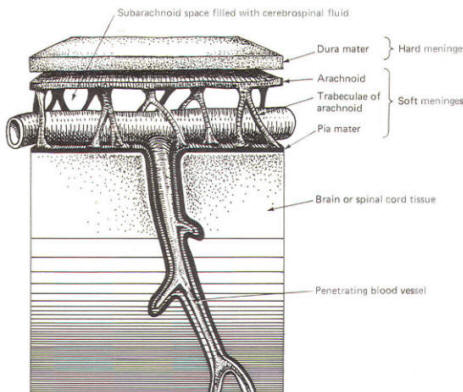 |
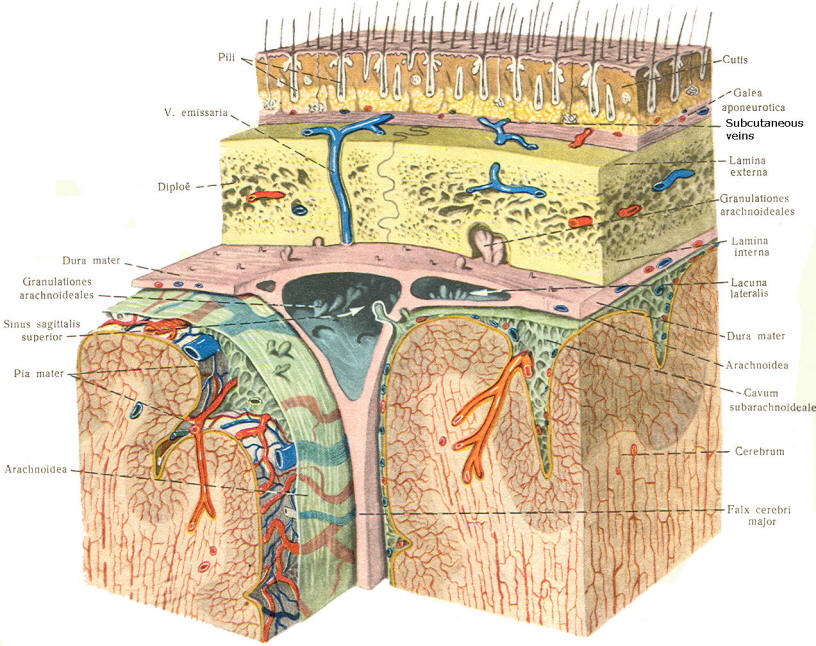 |
Fig-17 |
Fig-18 |
The
Ventricular System and the Cerebrospinal Fluid
The brain's
ventricular system develops right along with the brain itself.
The ventricles represent the full development of the lumen of
the cephalic end of the neural tube. They are filled with CSF,
interconnected, and continuous with the central canal of the
spinal cord. As the telencephalon develops in a lateral and
caudal direction, forming the hemispheres, the lumen of the
telencephalic neural tube develops along with them, forming two
lateral ventricles, one within each hemisphere. The CSF of the
two lateral ventricles eventually becomes separated by a thin
membrane, the septum pellucidum.
As the
diencephalon develops, the neural tube lumen expands in the
midsagittal plane into a broad, flat third ventricle. The CSF
in the lateral ventricles communicates with that in the third
ventricle through the foramina of Monro. The medial walls of the
diencephalon are completely bathed by the CSF of the third
ventricle, which flows around the interthalamic adhesion into
the cerebral aqueduct (Fig-19).
The
cerebral aqueduct is a narrow channel through the midbrain. The
CSF of the third ventricle flows through this channel into the
fourth ventricle located posterior to the pons and anterior to
the cerebellum. A small amount of the CSF from the fourth
ventricle flows into the central canal of the spinal cord, but
the greatest bulk of it flows into the subarachnoid spaces of
the meninges through three openings in the fourth ventricle. Two
of these openings, the foramina of Luschka, are located in the
anterior lateral extensions of the fourth ventricle around the
brainstem inferior to the pons. The other is the foramen of Magendie, a posterior opening in the inferior medullary velum below
the cerebellum.
Cerebrospinal Fluid Formation and Circulation
A
convoluted network of blood vessels traveling in the pia
projects into the ventricular system at several points. These
blood vessels are covered with ependyma since the entire
ventricular system is lined with ependyma. The vascular
protrusions with their coverings of specialized ependymal cells
are the choroid plexuses of the ventricular system (Fig-20). The choroid
plexus is located in the medial walls of the lateral ventricles,
the roof of the third ventricle, and the roof and anterior
lateral extensions of the fourth ventricle.
Cerebrospinal fluid is a clear and colorless liquid. It is
actively secreted by the choroid plexus into the ventricular
system at several points, but it is not a simple ultrafiltrate
of the plasma from which it is formed. The CSF formed by the
choroid plexus of the lateral ventricles flows through the
foramina of Monro into the third ventricle, where it joins that
produced by the choroid plexus of the third ventricle. As
previously noted, it then leaves the third ventricle through the
cerebral aqueduct to enter the fourth ventricle, where, most of
it flows out
through the foramina of Luschka and Magendie into the
subarachnoid spaces of the meningeal system (Fig-21). Through
this system CSF flows over and
around the entire brain and spinal cord.
Because CSF
is constantly being formed by the choroid plexus (about 500 ml
per day) and entering the ventriculomeningeal system, it is
apparent that it must also be removed from the system at the
same rate in order to maintain the constant value of 150 ml
which is common in the adult. Small elevations of the arachnoid
(arachnoid granulations) into the superior sagittal and
transverse sinuses apparently function as one-way valves
allowing CSF to be reabsorbed back into the venous blood (Fig-22).
|
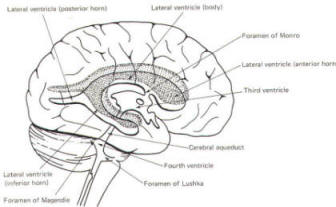 |
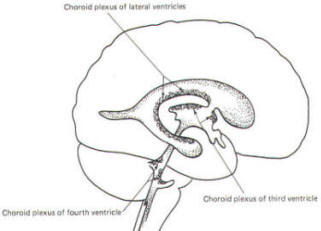 |
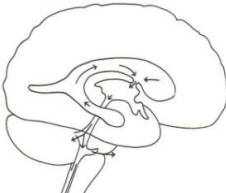 |
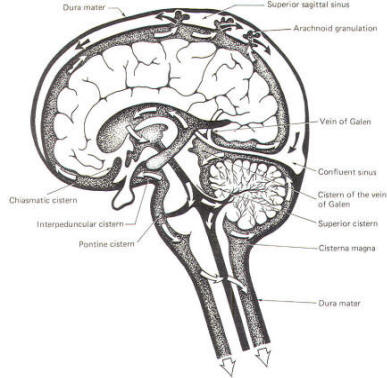 |
|
|
Fig-19 |
Fig-20 |
Fig-21 |
Fig-22 |
|
CSF tends
to accumulate in certain large subarachnoid areas of the brain
and spinal cord. These are called cisterns in the brain (Fig-13). The cisterna magna, just below the cerebellum, is the
largest of these. Others include the pontine cistern,
anterolateral to the pontomedullary border, the interpeduncular
cistern, anterior to the midbrain and inferior to the
diencephalon, the chiasmatic cistern surrounding the optic
chiasm, the superior cistern, between the cerebellum and the
inferior colliculi of the posterior midbrain, and the cistern of
the vein of Galen, posterior to the diencephalon. The
subarachnoid space is relatively large below the level of the
second lumbar vertebra and contains a relatively large amount of
CSF. Because this is below the
termination of the spinal cord, a needle can be safely passed
into the subarachnoid space in this region to withdraw a CSF
sample or to make an injection. Less commonly the cisterna
magna is used for this purpose.
Cerebrospinal Fluid Composition
The
chemical composition of CSF is similar to plasma. This is not
surprising since it is actively secreted by the choroid plexus.
The principal difference is that plasma contains about 300 times
as much protein as CSF. A brief summary of plasma and CSF
concentrations is given in Table-1.
Table-1
Plasma and CSF Concentrations |
|
Cerebrospinal fluid |
Plasma
|
Na+ |
147 meq/kg H2O
|
150 meq/kg
H2O
|
K+ |
2.9 meq/kg H2O
|
4.6 meq/kg
H2O
|
CI- |
113 meq/kg H2O
|
106 meq/kg
H2O
|
Osmolality |
289 mosmol/kg H2O
|
289 mosmol/kg
H2O
|
Protein |
20 mg/100 mL
|
6000 mg/100 mL |
Glucose |
64 mg/100 mL
|
100 mg/100 mL
|
pH |
7.307 |
7.397
|
|